Extracellular vesicles in pancreatic cancer: a new era in precision medicine
Introduction
Pancreatic cancer (PC) remains one of the most aggressive malignancies mostly due to the lack of early detection and poor response to surgical and chemotherapeutic therapies. Surgical excision is the definitive treatment option when diagnosed early, whereas chemotherapy and radiotherapy are used in the adjuvant, neoadjuvant or palliative setting (1). Nonetheless, cancer recurrence is common after surgical intervention (2,3). The growth of a tumour is mediated by the interaction between cancer cells and the neighbouring cells in the microenvironment and distant organs (4). This could be mediated by direct cell-cell interactions, secretory proteins, and extracellular vesicles (EVs). Precision medicine also called “personalised medicine” allows a tailored approach to an individual patient based on their risk profile and predicted response to therapy. Recent advancement in precision medicine has opened new avenues for therapeutic strategies, and one such emerging field in cancer research is the role of EVs. The EVs are described as lipid bilayer vesicles that are spontaneously released into the extracellular milieu by cells and lack the ability to replicate (5). They exhibit a broad spectrum of sizes, biogenesis, metabolic composition, and function, such as intercellular communication, immunological regulation, and disease progression. Based on the vesicle sizes and origin, EVs are often categorised into three categories: small EVs (sEVs) (30–150 nm), microvesicles (microparticles or ectosome, 50–1,000 nm), and apoptotic bodies (500–1,000 nm). Although EV release is a natural cellular process, a rapid increase in their release and a shift in their cargo’s constituents, such as DNA, RNA, microRNA (miRNA), and proteins, can promote the development of cancer. sEVs are produced by tumour cells and adjacent stromal cells, such as cancer-associated fibroblasts (CAFs), tumour-associated macrophages (TAMs), and bone marrow mesenchymal stem cells, during tumorigenesis. Furthermore, EVs carry biological cargo that influences tubule formation and cell proliferation, thus facilitating cancer growth (6,7) (Figure 1). Understanding the role of EVs in PC will open the door to revolutionising precision medicine by providing novel insight into this disease’s signatures, thus identifying potential therapeutic targets to improve patient outcomes, which we hope will be addressed by this mini review.
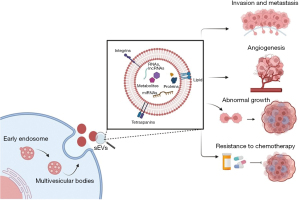
Role of PC-derived EVs in cancer progression
PC-derived EVs modulate angiogenesis
EVs are membrane-bound structures that are released by various cell types, including cancer cells (8). They contain various types of biomolecules, such as proteins, RNA, and lipids, which can modulate the function of neighbouring cells. In the context of PC, EVs have been shown to play a crucial role in regulating angiogenesis, the process by which new blood vessels form from existing ones. PC is known for its aggressive growth and poor prognosis, and the presence of new blood vessels is a hallmark of tumour progression. These new blood vessels help cancer cells to receive oxygen and nutrients to support their growth and spread to other parts of the body. Therefore, controlling angiogenesis has become an important target for the treatment of PC. Studies have shown that EVs derived from PC cells can modulate angiogenesis by affecting the expression of genes involved in this process. They can communicate with neighbouring cells and activate pathways that promote or suppress the formation of new blood vessels, depending on the specific composition and quantity of EVs released by the cancer cells. In addition, EVs can also carry signalling molecules that can promote tumour growth, such as vascular endothelial growth factor (VEGF), which is a well-known angiogenic factor. PC-derived EVs stimulate human umbilical vein endothelial cells (HUVECs) cell growth, migration, and pro-angiogenic factor production (9). Studies suggest that the expression of CD151 and tetraspanin 8 (Tspan8) in PC contributes to tumour growth (10). Further, overexpression of D6.1A in PC increases angiogenesis in vivo by transferring D6.1A to endothelial cells (11). This stimulates the expression of angiogenic proteins such as CD31 and VEGF. Abnormalities in wound healing and tube formation have been observed in mice lacking the proteins, Tspan8 and CD151, with severe consequences reported in double-knockout mice (10,12). Interestingly, Tspan8/CD151-containing serum-derived sEVs can alleviate these events in genetically modified animals by promoting tube formation through signalling pathways involving receptor tyrosine kinases (RTKs) and G protein-coupled receptors (GPCRs), eventually contributing to tumorigenesis in vivo. These findings suggest that blocking proteins such as Tspan8 and CD151 may have potential benefits in cancer therapy, as they play a role in tumour growth and development. The PC sEVs-derived miR-27a is found to significantly enhance angiogenesis in tumour-associated cells (13,14). This is achieved by increasing the production of proteins such as VEGFR, VEGF, matrix metalloproteinase 9 and matrix metalloproteinase 2. These proteins are involved in angiogenesis and cell migration. Additionally, annexin A1 (ANXA1) found in sEV cargo promotes endothelial tubular development and increases the motility of fibroblast and endothelial cells. Furthermore, the PKH-45H derived from sEVs has been found to induce angiogenesis in vitro. The angiogenic effect is mediated through the activation of specific molecular signalling pathways called extracellular signal-regulated kinase (ERK) and Akt, as well as a process called dynamin-dependent endocytosis. Additionally, tumour-derived sEVs improved the permeability of endothelial cells by transporting circular RNA molecules called circ-IARS into neighbouring cells (15). This triggers a chain of events inside the PC cells, resulting in tumour spread. Depleting myoferlin protein in PC cells has been shown to affect the production of sEVs and their transport to endothelial cells, resulting in reduced cell proliferation and propagation (16). Recent research revealed that cell signalling pathways involving DUSP2 and VEGF-C play a role in PC lymph angiogenesis and tumour invasion (17). Inhibiting DUSP2 increased the VEGF-C expression in PC-derived sEVs. In the PC animal model, VEGF-C-containing sEVs promote the proliferation of lymphatic endothelial cells and invasion of lymphatic vessels. In conclusion, the study of PC-derived EVs and their role in regulating angiogenesis has important implications for the understanding and treatment of this aggressive cancer. Further research in this area may lead to the development of new therapeutic strategies that can effectively control angiogenesis and prevent tumour progression.
PC-derived sEVs promote chemoresistance
Despite advances in chemotherapy drugs and treatment strategies, many patients with PC eventually develop resistance to these drugs, leading to treatment failure and poor outcomes. Understanding the mechanisms behind chemotherapy resistance is crucial for implementing effective chemotherapy regimens and future drug development. Recent studies have shown that EVs, specifically sEVs, play a significant role in the development of chemotherapy resistance in PC (18). The sEVs derived from chemotherapy-resistant PC cells have been shown to promote resistance in other cancer cells by delivering signalling molecules and miRNAs. These signalling molecules and miRNAs can suppress the expression of genes involved in the cellular response to chemotherapy drugs. This suppression can reduce the effectiveness of chemotherapy drugs, enabling PC cells to survive and continue to grow. Additionally, sEVs can also stimulate the activation of signalling pathways that promote the survival and proliferation of cancer cells, making them more resistant to chemotherapy. They can carry cancer-promoting molecules, such as oncogenes, to other parts of the body, where they can seed new tumours and contribute to disease progression. This process can also contribute to the development of chemotherapy resistance, as the new tumours may have different drug sensitivities than the primary tumour. One approach being explored to overcome chemotherapy resistance in PC is the inhibition of sEVs release from PC cells (19). By blocking the production of sEVs, it may be possible to minimise the effect of chemoresistance and reduce the risk of relapse. Chemoresistance develops after prolonged exposure to chemotherapeutic drugs. The chemotherapy drug such as, gemcitabine (GEM) alters tumour cell cargo, including sEVs. MiR-155 is an onco-miRNA that has been reported to be significantly expressed in the majority of PC cases and to correlate with poor PC patient survival (20). In in vitro experiments and nonobese diabetic/severe combined immunodeficiency (NOD/SCID) animals, GEM-induced overexpression of miR-155 in PC cells derived sEVs which can prevent GEM-induced apoptosis in other PC cells. Deoxycytidine kinase (DCK) is one of the targets that miR-155 aims to affect. During chemotherapy DCK has the ability to convert GEM into an active form that incorporates into DNA, hindering its replication and synthesis, causing tumour associated cell death. MiR-155 in PC derived sEVs downregulates DCK expression and enhances PC cell survival (21). Following exosomal miR-155 absorption, a pro-apoptotic p53 effector gene known as TP53INP1 was observed to have a decreased expression level in PC cells. Furthermore, miR-155 overexpression serves as a tumour defence mechanism against chemotherapeutic drugs, indicating a poor prognosis. This phenomenon is further exemplified by the EphA2 protein, as chemo-resistant PC cells produce sEVs enriched with EphA2, which are then delivered to nearby PC cells to assist in their survival (22). GEM upregulates the expression of sEV-derived miR210, which can be delivered from chemoresistant to chemosensitive PC cells (23). In conclusion, sEVs play a critical role in the development of chemotherapy resistance in PC. Understanding the mechanisms by which these vesicles promote resistance is crucial for improving patient outcomes. Future research in this area may lead to the development of new therapeutic strategies that can effectively target sEVs and overcome chemotherapy resistance in PC.
PC-derived sEVs in tumour proliferation and progression
The liver is the most common site for PC metastases. The physical location of the liver adjacent to the pancreas and the vascular supply enables tumour seeding via the portal vein and makes it a common metastatic site. Several research teams have investigated the involvement of EVs in enhancing the metastatic cascade in PC (24,25). One of the research teams has identified that PC-derived EVs trigger pre-metastatic niche formation in the liver, which increases the tumour load (26). Kupffer cells are responsible for the delivery of PC-derived EVs that contain the macrophage migration inhibitory factor (MIF) (27). These EVs can alter the hepatic milieu by triggering an inflammatory reaction and generating fibrotic pathways. These modifications promote tumour progression in the liver by encouraging liver cells to take up immune cells and enhance transforming growth factor (TGF) and fibronectin synthesis. Furthermore, PC-derived EVs supplemented in integrin v5 tend to migrate to the liver, whereas EVs enriched in integrins 64 and 61 are identified in the lungs (28). This selectivity of PC-derived EVs targeting distant sites, notably the liver, indicates that EVs are significant mediators of tumour proliferation and progression. More research is needed to comprehend the molecular pathways underlying distant site metastasis regulated by PC-derived EVs.
EVs vs. cancer biomarkers
Scientific efforts are being made to detect early symptoms of the disease in high-risk individuals. This is particularly important in PC, where understanding the disease tumorigenesis, monitoring therapy, and predicting the progress is essential. High risk individual comprises of family history, genetic mutation associated with PC, suffering from diabetes and chronic pancreatitis. Given the asymptomatic onset of PC, the paramount focus lies on early detection using reliable biomarkers. Minimally invasive biomarkers such as cell-free DNA and circulating tumour cells have yield promising results (29,30). These biomarkers, however, pose a challenge for large-scale applications due to their low concentration in blood plasma, difficult isolation techniques, and long-term storage and handling challenges. Moreover, EV cargo-derived RNA is considered to be more reliable than cell-free DNA as they are resistant to degradation (31). In addition, EVs are comparatively highly concentrated in the blood and EV-derived cargo is unique to disease type (32,33).
In order to fully harness the diagnostic and prognostic potential of EVs in PC, it is imperative that practical challenges need to be addressed. The variability in isolation of EVs and contamination of albumin in plasma/serum samples can affect downstream application of EVs. Utilisation of different isolation techniques may result in varying EV concentration, which consequently impacts the purity and yield of retrieved EVs. Further, EVs composition can evolve according to the pathological and physiological conditions of PC. Future studies need to focus on EV expression based on tumour staging and detecting PC in early stages. Additionally, there is a need to increase the sample size based on high-risk population, chronic pancreatitis/benign pancreatic conditions and existing genetic predisposition (Table S1 provides a comprehensive list of potential EVs, highlighting their advantages and disadvantages in comparison to other biomarkers).
Role of EVs as potential PC biomarkers
Due to a lack of early symptoms, screening tools, and biomarkers, 80% of PC patients are identified at a late stage. Currently, carbohydrate antigen 19-9 (CA19-9) is the only biomarker available for PC (34); However, its limited sensitivity makes it unsuitable for early PC detection. As a result, innovative early diagnostic tools are crucial to identify PC patients at an early stage for treatment, thus having an impact on overall survival. Given the importance of EVs released by cancer cells in PC progression, the functional cargo carried by EVs shows potential as biomarkers for PC (Table S2). Using EV as an early diagnostic tool in PC has its advantages. The EVs are ubiquitously present in nearly all body fluids (such as serum, saliva, urine) making them easily accessible. Further, the changes in abundance and cargo profile of PC-derived EVs would make them applicable to detect early stages of cancer before the onset of symptoms. This will allow room for screening at-risk populations such as those presenting with chronic pancreatitis, family history or other PC hallmarks (5). In addition to early cancer detection, EV cargo can be used to monitor disease progression and treatment response thus allowing for patient stratification based on progression, treatment sensitivity and response. There are also promising studies being conducted to develop EV-based detection tests for PC that can be used in clinical settings (35,36). These tests have the potential to be highly precise for PC detection, offering a non-invasive and reliable method for disease monitoring. Several research teams have isolated and collected. EVs from the sample of PC patients and healthy controls and assessed specific miRNA-derived EV content using reverse transcription polymerase chain reaction (RT-PCR) and miRNA-specialised sensors to determine if EVs can be used as diagnostic tool (37-42). MiR-550, miR-21 miR-17-5p and miR-10b expression was found to be elevated in PC patients, implying that they can serve as potential markers for PC detection (41,43-46). Madhavan et al. examined blood EVs for five PC-initiating cell markers and four combination miRNAs (47). Comparing PC-initiating cell signatures and miRNA EV markers may facilitate PC detection and differentiation from healthy adults. Proteomic investigation of EVs obtained from pancreatic juice of PC patients identified possible diagnostic biomarkers including mucins (MUCs), cystic fibrosis transmembrane conductance regulator (CFTR), and multidrug resistance 1 (MDR1) proteins. However, these biomarkers are not confined to PC-derived sEVs (48-50). Further, a research team developed PC cancer mice model by implanting Panc02. They could suppress the secretions of PC derive sEVs by transfecting with dominant negative Rab-11 (51). This resulted in the transcriptome detection of salivary sEVs (DN-Rab11). Within malignant mouse saliva, 209 differently increased transcriptomic marker genes were discovered, with six proven to overexpress in PC peripheral blood and saliva derived sEVs, beta-carotene oxygenase 31, Apbb1ip, Daf2, FoxP1, GnG2, and Incenp (51). Interestingly, Apbb1ip a gene involved in actin structuring, has been found to be elevated in mice deficient in PC sEVs. However, the increase in gene expression is lower in these mice compared to the PC sEVs mouse model, which suggests that this gene may not be present solely in sEVs. Combining multiple biomarkers may increase specificity and sensitivity in cancer detection, as one constituent of sEV cargo might not prove to be sufficient to detect PC in at-risk patients. Glypican-1 (GPC-1) is a type of heparan sulphate proteoglycan present on cell membranes and in the extracellular matrix, where it signals cell growth and proliferation (33,52).
Role of EVs as a therapeutic tool
Rapid advancements in therapeutic tools have ushered in a new era of treatment strategies, where both biological and artificial vehicles are being harnessed for enhanced therapeutic outcomes (53-55). These remarkable tools share certain key physiological characteristics, such as their nanoscopic size and their capacity to efficiently deliver drugs to specific targets. Among these therapeutic tools, sEVs have emerged as particularly promising agents due to their unique capabilities (56). Notably, sEVs have demonstrated the remarkable ability to traverse the formidable blood-brain barrier, allowing for precise drug delivery to targeted sites. Unlike the artificial nanoparticles, the sEVs will not be eliminated by phagocytic system. sEVs constitute of range of proteins that help in bypassing immunological response. This intrinsic ability to elude immune detection makes sEVs an appealing choice for therapeutic interventions. Further, sEVs are comparatively more efficient as drug carriers than liposomes. As sEVs exhibit a distinctive characteristic of circulating in the bloodstream for prolonged time in comparison to liposomes. Moreover, sEVs offer distinct advantage as drug carrier by accommodating significantly higher quantities of drugs compared to traditional liposomes.
EVs can be used to transfer therapeutic molecules, such as RNA and miRNA, directly to cancer cells, which can alter the physiology of the cancer cells and improve treatment outcomes. This approach presents significant challenges such as maintaining the stability of the vehicles. Using therapeutic vehicles can be challenging due to the need for specific targeting mechanism, maintain stability of vehicles in the body, and avoid toxicity. The investigation of the application of EVs in therapies is still in its early phase (Table S3). In 2019, Batista and Melo published an assessment of the approaches that have been investigated (57). One of the approaches focused on inhibiting the biogenesis or the release of EVs, thereby restricting the cancer-promoting signalling from the EVs.
EVs as active drug delivery tool
EVs are known to transport unique biomolecules that are effectively internalised by target cells. Furthermore, EVs possess the unique characteristic to exhibit negligible toxicity and minimal immunogenicity, such characteristics can make EVs a compelling option for becoming a targeted therapeutic agent (58,59). One of the research teams discovered that by modifying EVs to target a specific gene mutation, were able to reduce tumour growth and increase lifespan of mice (60). This study focused on using EVs to target the oncogenic mutation; KRASG12D which resulted in a greater reduction in tumour size compared to liposomes loaded with the same siRNA or shRNA. After 200 days of therapy, KRAS and KRASG12D expression was decreased in malignant tumours and pancreas, which was believed to be the cause of the observed results. These findings could potentially lead to the development of new targeted cancer therapies. The intervention effectively reduced the proliferation of the KRASG12D mutation in mice. According to a study conducted by Pascucci et al., administration of paclitaxel to mesenchymal stromal cells resulted in secretion of EVs with cargo containing paclitaxel. These EVs inhibited PC cell growth in vitro (61). Subsequently, one of the scientific groups demonstrated the potential of employing EV loaded with paclitaxel as a treatment of multidrug-resistant cancer (62). Recent scientific efforts have engineered cells that can generate sEVs with a targeted surface protein content, including interleukin-12, targeted T- and natural killer-cells. The sEVs has the potential to be modified via the addition of a specific glycoprotein to enhance cellular function. Administering anticancer drugs can activate the stimulator of interferon gene (STING) pathway, which presents a promising strategy in the fight against cancer. However, further research is required to determine its effectiveness and potential clinical applications (63). EVs as therapeutic vehicles are promising area of research for the treatment of PC, and their continued development and optimisation holds great promise for improving the prognosis for PC patients.
Interrupting EV production and targeting molecular signalling
Chemoresistance is a key factor in the fight to treat PC effectively. GEM is currently one of the most utilised drugs. Unfortunately, the EVs produced following GEM therapy frequently cause an increased GEM resistance. Richards et al. reported in 2022 that GEM therapy significantly boosted the generation of EVs by CAFs (64). This proved EVs have a significant impact on PC progression and can be considered as a possible target for therapeutic intervention. Inhibiting EV secretion or uptake is widely considered a novel approach to PC therapy. Catalano et al. employed the drug GW4869 to inhibit the release of EVs harbouring miR-146a from CAFs (65). They observed that certain cell lines preserved their sensitivity to chemotherapy. Consequently, blocking EV uptake may elicit a suppressive impact on cancer cells. Research revealed that the protein REG3β released from pancreatic tissue interferes with EV uptake and internationalisation of the recipient cells, effectively restricting migration and metabolism in PC malignant cells (66). Further, PC cells treated with GEM enrich miR-155 expression and transfer miR-155 to other PC cells, inhibiting cell apoptosis. PC derived EVs possess the ability to mitigate the immune response by reducing the efficacy of antigen presentation. Depleting miRNAs from PC-derived EVs has been proven to increase cancer resistance in dendritic cells and other cells at distant sites (67). Interfering with EV production may reduce the effect of the tumour microenvironment and may enhance the efficacy of immunotherapy in PC. EVs have emerged as a promising tool for improving PC patients’ overall survival. By engineering EVs to carry pharmacological drug and specific surface modification may enable them to selectively recognise and bind to PC cells. The pharmaceutical drug may include chemotherapeutic agents, novel targeted therapies or siRNAs are designed to inhibit signalling pathways associated to PC progression. In conclusion, the PC derived EVs are small vesicles secreted by cells that can be engineered to deliver therapeutic agents directly to cancer cells. They can also traverse biological barriers and efficiently transport bioactive molecules that may interfere with the molecular mechanisms driving PC. Moreover, EVs can be analysed to provide diagnostic and prognostic value in PC. As research in EV-based therapies continues to evolve, it offers a beacon of hope for PC patients in need of more effective and tailored therapeutic options.
Future directions
PC is a challenging disease to diagnose and treat due to its advanced stage at presentation and systemic spread. The presence of EVs in bodily fluids such as urine, blood, pancreatic juice, and saliva makes them an ideal candidate as biomarkers. Current literature demonstrates the role of EVs biological cargo in PC progression, as they regulate tumour microenvironment, promote neo-angiogenesis and contribute to drug resistance (68). With the development of next-generation biotechnological tools such as single-cell and single-EV analysis, high-throughput, and shotgun proteomics, the capacity to assess possible candidate biomarkers may likely improve their utility further (69). The use of EVs in early diagnosis would provide an effective screening opportunity in high-risk individuals, leading to better outcomes for patients.
Conclusions
To summarize, EVs have great potential as biomarkers for PC. These bubble-like vesicles carry information related to advancement of the disease, therapy response and early detection. Nanoparticle-based EV analysis is a new technology that could enhance the sensitivity and specificity of EV-based diagnostic procedures and their usefulness as biomarkers. However, several obstacles must be solved before EVs may be used as a reliable biomarker for PC. These include increasing the specificity and sensitivity of current diagnostic tools, confirming the findings of more extensive clinical trials, and integrating EVs with existing diagnostic procedures such as imaging and biopsy to increase diagnosis accuracy. Despite the current challenges, ongoing research offers the potential to overcome these obstacles and make EVs a reliable biomarker in the fight against PC, ultimately improving patient survival rates.
Acknowledgments
Funding: None.
Footnote
Peer Review File: Available at https://tgh.amegroups.com/article/view/10.21037/tgh-23-53/prf
Conflicts of Interest: All authors have completed the ICMJE uniform disclosure form (available at https://tgh.amegroups.com/article/view/10.21037/tgh-23-53/coif). K.R. serves as an unpaid editorial board member of Translational Gastroenterology and Hepatology from April 2023 to March 2025. The other authors have no conflicts of interest to declare.
Ethical Statement: The authors are accountable for all aspects of the work in ensuring that questions related to the accuracy or integrity of any part of the work are appropriately investigated and resolved.
Open Access Statement: This is an Open Access article distributed in accordance with the Creative Commons Attribution-NonCommercial-NoDerivs 4.0 International License (CC BY-NC-ND 4.0), which permits the non-commercial replication and distribution of the article with the strict proviso that no changes or edits are made and the original work is properly cited (including links to both the formal publication through the relevant DOI and the license). See: https://creativecommons.org/licenses/by-nc-nd/4.0/.
References
- Springfeld C, Ferrone CR, Katz MHG, et al. Neoadjuvant therapy for pancreatic cancer. Nat Rev Clin Oncol 2023;20:318-37. [Crossref] [PubMed]
- Wang S, Zheng Y, Yang F, et al. The molecular biology of pancreatic adenocarcinoma: translational challenges and clinical perspectives. Signal Transduct Target Ther 2021;6:249. [Crossref] [PubMed]
- Gupta R, Amanam I, Chung V. Current and future therapies for advanced pancreatic cancer. J Surg Oncol 2017;116:25-34. [Crossref] [PubMed]
- Hamada S, Masamune A, Shimosegawa T. Alteration of pancreatic cancer cell functions by tumor-stromal cell interaction. Front Physiol 2013;4:318. [Crossref] [PubMed]
- Yáñez-Mó M, Siljander PR, Andreu Z, et al. Biological properties of extracellular vesicles and their physiological functions. J Extracell Vesicles 2015;4:27066. [Crossref] [PubMed]
- Moeng S, Son SW, Lee JS, et al. Extracellular Vesicles (EVs) and Pancreatic Cancer: From the Role of EVs to the Interference with EV-Mediated Reciprocal Communication. Biomedicines 2020;8:267. [Crossref] [PubMed]
- Jabalee J, Towle R, Garnis C. The Role of Extracellular Vesicles in Cancer: Cargo, Function, and Therapeutic Implications. Cells 2018;7:93. [Crossref] [PubMed]
- Xu R, Rai A, Chen M, et al. Extracellular vesicles in cancer - implications for future improvements in cancer care. Nat Rev Clin Oncol 2018;15:617-38. [Crossref] [PubMed]
- Guo Z, Wang X, Yang Y, et al. Hypoxic Tumor-Derived Exosomal Long Noncoding RNA UCA1 Promotes Angiogenesis via miR-96-5p/AMOTL2 in Pancreatic Cancer. Mol Ther Nucleic Acids 2020;22:179-95. [Crossref] [PubMed]
- Yue S, Mu W, Erb U, et al. The tetraspanins CD151 and Tspan8 are essential exosome components for the crosstalk between cancer initiating cells and their surrounding. Oncotarget 2015;6:2366-84. [Crossref] [PubMed]
- Gesierich S, Berezovskiy I, Ryschich E, et al. Systemic induction of the angiogenesis switch by the tetraspanin D6.1A/CO-029. Cancer Res 2006;66:7083-94. [Crossref] [PubMed]
- Zhao K, Erb U, Hackert T, et al. Distorted leukocyte migration, angiogenesis, wound repair and metastasis in Tspan8 and Tspan8/CD151 double knockout mice indicate complementary activities of Tspan8 and CD51. Biochim Biophys Acta Mol Cell Res 2018;1865:379-91. [Crossref] [PubMed]
- Pessolano E, Belvedere R, Bizzarro V, et al. Annexin A1 May Induce Pancreatic Cancer Progression as a Key Player of Extracellular Vesicles Effects as Evidenced in the In Vitro MIA PaCa-2 Model System. Int J Mol Sci 2018;19:3878. [Crossref] [PubMed]
- Novizio N, Belvedere R, Pessolano E, et al. Annexin A1 Released in Extracellular Vesicles by Pancreatic Cancer Cells Activates Components of the Tumor Microenvironment, through Interaction with the Formyl-Peptide Receptors. Cells 2020;9:2719. [Crossref] [PubMed]
- Li J, Li Z, Jiang P, et al. Circular RNA IARS (circ-IARS) secreted by pancreatic cancer cells and located within exosomes regulates endothelial monolayer permeability to promote tumor metastasis. J Exp Clin Cancer Res 2018;37:177. [Crossref] [PubMed]
- Blomme A, Fahmy K, Peulen O, et al. Myoferlin is a novel exosomal protein and functional regulator of cancer-derived exosomes. Oncotarget 2016;7:83669-83. [Crossref] [PubMed]
- Wang CA, Chang IH, Hou PC, et al. DUSP2 regulates extracellular vesicle-VEGF-C secretion and pancreatic cancer early dissemination. J Extracell Vesicles 2020;9:1746529. Erratum in: J Extracell Vesicles 2020;9:i. [Crossref] [PubMed]
- Słomka A, Kornek M, Cho WC. Small Extracellular Vesicles and Their Involvement in Cancer Resistance: An Up-to-Date Review. Cells 2022;11:2913. [Crossref] [PubMed]
- Hayatudin R, Fong Z, Ming LC, et al. Overcoming Chemoresistance via Extracellular Vesicle Inhibition. Front Mol Biosci 2021;8:629874. [Crossref] [PubMed]
- Khan MA, Zubair H, Srivastava SK, et al. Insights into the Role of microRNAs in Pancreatic Cancer Pathogenesis: Potential for Diagnosis, Prognosis, and Therapy. Adv Exp Med Biol 2015;889:71-87. [Crossref] [PubMed]
- Patel GK, Khan MA, Bhardwaj A, et al. Exosomes confer chemoresistance to pancreatic cancer cells by promoting ROS detoxification and miR-155-mediated suppression of key gemcitabine-metabolising enzyme, DCK. Br J Cancer 2017;116:609-19. [Crossref] [PubMed]
- Fan J, Wei Q, Koay EJ, et al. Chemoresistance Transmission via Exosome-Mediated EphA2 Transfer in Pancreatic Cancer. Theranostics 2018;8:5986-94. [Crossref] [PubMed]
- Silakit R, Kitirat Y, Thongchot S, et al. Potential role of HIF-1-responsive microRNA210/HIF3 axis on gemcitabine resistance in cholangiocarcinoma cells. PLoS One 2018;13:e0199827. [Crossref] [PubMed]
- Yang J, Zhang Z, Zhang Y, et al. ZIP4 Promotes Muscle Wasting and Cachexia in Mice With Orthotopic Pancreatic Tumors by Stimulating RAB27B-Regulated Release of Extracellular Vesicles From Cancer Cells. Gastroenterology 2019;156:722-734.e6. [Crossref] [PubMed]
- Sagar G, Sah RP, Javeed N, et al. Pathogenesis of pancreatic cancer exosome-induced lipolysis in adipose tissue. Gut 2016;65:1165-74. [Crossref] [PubMed]
- Costa-Silva B, Aiello NM, Ocean AJ, et al. Pancreatic cancer exosomes initiate pre-metastatic niche formation in the liver. Nat Cell Biol 2015;17:816-26. [Crossref] [PubMed]
- Costa Verdera H, Gitz-Francois JJ, Schiffelers RM, et al. Cellular uptake of extracellular vesicles is mediated by clathrin-independent endocytosis and macropinocytosis. J Control Release 2017;266:100-8. [Crossref] [PubMed]
- Hoshino A, Costa-Silva B, Shen TL, et al. Tumour exosome integrins determine organotropic metastasis. Nature 2015;527:329-35. [Crossref] [PubMed]
- Trapp E, Janni W, Schindlbeck C, et al. Presence of Circulating Tumor Cells in High-Risk Early Breast Cancer During Follow-Up and Prognosis. J Natl Cancer Inst 2019;111:380-7. [Crossref] [PubMed]
- Zhao X, Ma Y, Dong X, et al. Molecular characterization of circulating tumor cells in pancreatic ductal adenocarcinoma: potential diagnostic and prognostic significance in clinical practice. Hepatobiliary Surg Nutr 2021;10:796-810. [Crossref] [PubMed]
- Malentacchi F, Pizzamiglio S, Verderio P, et al. Influence of storage conditions and extraction methods on the quantity and quality of circulating cell-free DNA (ccfDNA): the SPIDIA-DNAplas External Quality Assessment experience. Clin Chem Lab Med 2015;53:1935-42. [Crossref] [PubMed]
- Nanou A, Miao J, Coumans FAW, et al. Tumor-Derived Extracellular Vesicles as Complementary Prognostic Factors to Circulating Tumor Cells in Metastatic Breast Cancer. JCO Precis Oncol 2023;7:e2200372. [Crossref] [PubMed]
- Buscail E, Chauvet A, Quincy P, et al. CD63-GPC1-Positive Exosomes Coupled with CA19-9 Offer Good Diagnostic Potential for Resectable Pancreatic Ductal Adenocarcinoma. Transl Oncol 2019;12:1395-403. [Crossref] [PubMed]
- Lee T, Teng TZJ, Shelat VG. Carbohydrate antigen 19-9 - tumor marker: Past, present, and future. World J Gastrointest Surg 2020;12:468-90. [Crossref] [PubMed]
- Lener T, Gimona M, Aigner L, et al. Applying extracellular vesicles based therapeutics in clinical trials - an ISEV position paper. J Extracell Vesicles 2015;4:30087. [Crossref] [PubMed]
- Zhao Z, Fan J, Hsu YS, et al. Extracellular vesicles as cancer liquid biopsies: from discovery, validation, to clinical application. Lab Chip 2019;19:1114-40. [Crossref] [PubMed]
- Wang C, Wang J, Cui W, et al. Serum Exosomal miRNA-1226 as Potential Biomarker of Pancreatic Ductal Adenocarcinoma. Onco Targets Ther 2021;14:1441-51. [Crossref] [PubMed]
- Verel-Yilmaz Y, Fernández JP, Schäfer A, et al. Extracellular Vesicle-Based Detection of Pancreatic Cancer. Front Cell Dev Biol 2021;9:697939. [Crossref] [PubMed]
- Takahasi K, Iinuma H, Wada K, et al. Usefulness of exosome-encapsulated microRNA-451a as a minimally invasive biomarker for prediction of recurrence and prognosis in pancreatic ductal adenocarcinoma. J Hepatobiliary Pancreat Sci 2018;25:155-61. [Crossref] [PubMed]
- Robless EE, Howard JA, Casari I, et al. Exosomal long non-coding RNAs in the diagnosis and oncogenesis of pancreatic cancer. Cancer Lett 2021;501:55-65. [Crossref] [PubMed]
- Que R, Ding G, Chen J, et al. Analysis of serum exosomal microRNAs and clinicopathologic features of patients with pancreatic adenocarcinoma. World J Surg Oncol 2013;11:219. [Crossref] [PubMed]
- Marin AM, Mattar SB, Amatuzzi RF, et al. Plasma Exosome-Derived microRNAs as Potential Diagnostic and Prognostic Biomarkers in Brazilian Pancreatic Cancer Patients. Biomolecules 2022;12:769. [Crossref] [PubMed]
- Taller D, Richards K, Slouka Z, et al. On-chip surface acoustic wave lysis and ion-exchange nanomembrane detection of exosomal RNA for pancreatic cancer study and diagnosis. Lab Chip 2015;15:1656-66. [Crossref] [PubMed]
- Joshi GK, Deitz-McElyea S, Liyanage T, et al. Label-Free Nanoplasmonic-Based Short Noncoding RNA Sensing at Attomolar Concentrations Allows for Quantitative and Highly Specific Assay of MicroRNA-10b in Biological Fluids and Circulating Exosomes. ACS Nano 2015;9:11075-89. [Crossref] [PubMed]
- Vannini I, Rossi T, Melloni M, et al. Analysis of EVs from patients with advanced pancreatic cancer identifies antigens and miRNAs with predictive value. Mol Ther Methods Clin Dev 2023;29:473-82. [Crossref] [PubMed]
- Roy JW, Wajnberg G, Ouellette A, et al. Small RNA sequencing analysis of peptide-affinity isolated plasma extracellular vesicles distinguishes pancreatic cancer patients from non-affected individuals. Sci Rep 2023;13:9251. [Crossref] [PubMed]
- Madhavan B, Yue S, Galli U, et al. Combined evaluation of a panel of protein and miRNA serum-exosome biomarkers for pancreatic cancer diagnosis increases sensitivity and specificity. Int J Cancer 2015;136:2616-27. [Crossref] [PubMed]
- Lu Z, Kleeff J, Shrikhande S, et al. Expression of the multidrug-resistance 1 (MDR1) gene and prognosis in human pancreatic cancer. Pancreas 2000;21:240-7. [Crossref] [PubMed]
- Liu H, Wu W, Liu Y, et al. Predictive value of cystic fibrosis transmembrane conductance regulator (CFTR) in the diagnosis of gastric cancer. Clin Invest Med 2014;37:E226-32. [Crossref] [PubMed]
- Jonckheere N, Skrypek N, Van Seuningen I. Mucins and pancreatic cancer. Cancers (Basel) 2010;2:1794-812. [Crossref] [PubMed]
- Lau C, Kim Y, Chia D, et al. Role of pancreatic cancer-derived exosomes in salivary biomarker development. J Biol Chem 2013;288:26888-97. [Crossref] [PubMed]
- Melo SA, Luecke LB, Kahlert C, et al. Glypican-1 identifies cancer exosomes and detects early pancreatic cancer. Nature 2015;523:177-82. [Crossref] [PubMed]
- Higa LH, Jerez HE, de Farias MA, et al. Ultra-small solid archaeolipid nanoparticles for active targeting to macrophages of the inflamed mucosa. Nanomedicine (Lond) 2017;12:1165-75. [Crossref] [PubMed]
- Martínez-Carmona M, Lozano D, Colilla M, et al. Lectin-conjugated pH-responsive mesoporous silica nanoparticles for targeted bone cancer treatment. Acta Biomater 2018;65:393-404. [Crossref] [PubMed]
- Pinese C, Lin J, Milbreta U, et al. Sustained delivery of siRNA/mesoporous silica nanoparticle complexes from nanofiber scaffolds for long-term gene silencing. Acta Biomater 2018;76:164-77. [Crossref] [PubMed]
- El Andaloussi S, Lakhal S, Mäger I, et al. Exosomes for targeted siRNA delivery across biological barriers. Adv Drug Deliv Rev 2013;65:391-7. [Crossref] [PubMed]
- Batista IA, Melo SA. Exosomes and the Future of Immunotherapy in Pancreatic Cancer. Int J Mol Sci 2019;20:567. [Crossref] [PubMed]
- Vader P, Mol EA, Pasterkamp G, et al. Extracellular vesicles for drug delivery. Adv Drug Deliv Rev 2016;106:148-56. [Crossref] [PubMed]
- Johnsen KB, Gudbergsson JM, Skov MN, et al. A comprehensive overview of exosomes as drug delivery vehicles - endogenous nanocarriers for targeted cancer therapy. Biochim Biophys Acta 2014;1846:75-87. [PubMed]
- Kamerkar S, LeBleu VS, Sugimoto H, et al. Exosomes facilitate therapeutic targeting of oncogenic KRAS in pancreatic cancer. Nature 2017;546:498-503. [Crossref] [PubMed]
- Pascucci L, Coccè V, Bonomi A, et al. Paclitaxel is incorporated by mesenchymal stromal cells and released in exosomes that inhibit in vitro tumor growth: a new approach for drug delivery. J Control Release 2014;192:262-70. [Crossref] [PubMed]
- Kim MS, Haney MJ, Zhao Y, et al. Development of exosome-encapsulated paclitaxel to overcome MDR in cancer cells. Nanomedicine 2016;12:655-64. [Crossref] [PubMed]
- Jang SC, Economides KD, Moniz RJ, et al. ExoSTING, an extracellular vesicle loaded with STING agonists, promotes tumor immune surveillance. Commun Biol 2021;4:497. [Crossref] [PubMed]
- Richards KE, Xiao W, Hill R, et al. Cancer-Associated Fibroblasts Confer Gemcitabine Resistance to Pancreatic Cancer Cells through PTEN-Targeting miRNAs in Exosomes. Cancers (Basel) 2022;14:2812. [Crossref] [PubMed]
- Catalano M, O'Driscoll L. Inhibiting extracellular vesicles formation and release: a review of EV inhibitors. J Extracell Vesicles 2020;9:1703244. [Crossref] [PubMed]
- Bonjoch L, Gironella M, Iovanna JL, et al. REG3β modifies cell tumor function by impairing extracellular vesicle uptake. Sci Rep 2017;7:3143. [Crossref] [PubMed]
- Javeed N, Gustafson MP, Dutta SK, et al. Immunosuppressive CD14(+)HLA-DR(lo/neg) monocytes are elevated in pancreatic cancer and "primed" by tumor-derived exosomes. Oncoimmunology 2017;6:e1252013. [Crossref] [PubMed]
- Emmanouilidi A, Paladin D, Greening DW, et al. Oncogenic and Non-Malignant Pancreatic Exosome Cargo Reveal Distinct Expression of Oncogenic and Prognostic Factors Involved in Tumor Invasion and Metastasis. Proteomics 2019;19:e1800158. [Crossref] [PubMed]
- Altelaar AF, Munoz J, Heck AJ. Next-generation proteomics: towards an integrative view of proteome dynamics. Nat Rev Genet 2013;14:35-48. [Crossref] [PubMed]
Cite this article as: Panda A, Falasca M, Ragunath K. Extracellular vesicles in pancreatic cancer: a new era in precision medicine. Transl Gastroenterol Hepatol 2024;9:29.